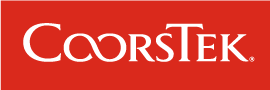
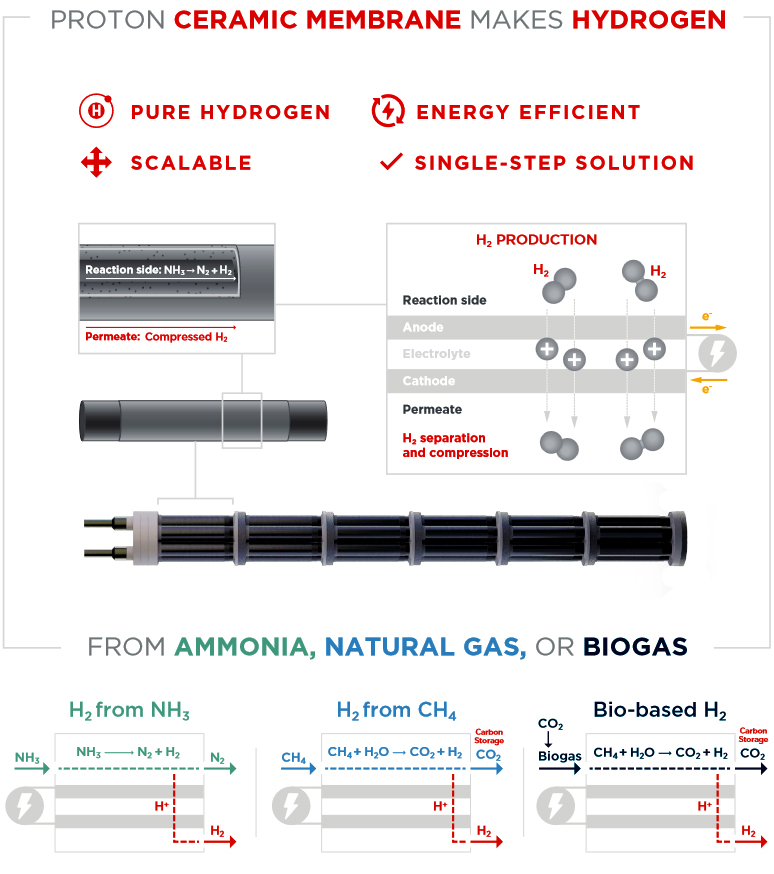
Single-step
hydrogen production from NH3, CH4, and biogas in stacked proton
ceramic reactors
DANIEL CLARK, HARALD
MALEROD-FUELD,
MICHAEL BUDD
,
IRENE YUSTE-TIRADOS,
DUSTIN
BEEAFF,
SIMEN
AAMODT,
KEVIN NGUYEN,
LUCA
ANSALONI,
THIJS
PETERS,
CHRISTIAN
KJOLSETH +8
authors
SCIENCE
21 April 2022
Volume 376,Issue 6591 pp.390-393
Electrifying
membranes to deliver hydrogen
Sourcing hydrogen with ceramic reactors
An alternative to directly
transporting hydrogen produced at large scales through steam reforming
for applications such as vehicular fueling is smaller scale, on-site
production from methane or carriers such as ammonia. The hydrogen
produced must be separated from co-produced carbon dioxide or
nitrogen. Proton ceramic electrochemical reactors can extract pure
hydrogen from gas mixtures by electrolytically pumping protons across
the membrane at 800°C, but as the extraction proceeds, temperature
gradients and entropic effects lead to efficiency drops. Clark et al.
developed a nickel-based glass-ceramic composite interconnect that
allowed for the design of a more complex reactor pathway (see the
Perspective by Shih and Haile). Counterflowing streams balanced heat
flows and maintained stable operating conditions that enabled 99%
efficiency of hydrogen recovery. -PDS
Abstract
Proton ceramic reactors
offer efficient extraction of hydrogen from ammonia, methane, and
biogas by coupling endothermic reforming reactions with heat from
electrochemical gas separation and compression. Preserving this
efficiency in scale-up from cell to stack level poses challenges to
the distribution of heat and gas flows and electric current throughout
a robust functional design. Here, we demonstrate a 36-cell
well-balanced reactor stack enabled by a new interconnect that
achieves complete conversion of methane with more than 99% recovery
to pressurized hydrogen, leaving a concentrated stream of carbon
dioxide. Comparable cell performance was also achieved with ammonia,
and the operation was confirmed at pressures exceeding 140 bars. The
stacking of proton ceramic reactors into practical
thermo-electrochemical devices demonstrates their potential in
efficient hydrogen production.
Hydrogen can be produced from CH i-rich
streams through steam reforming and water-gas shift (SMR+WGS, CH4
+ 2H2O =CO2+ 4H2, llrxnH°
= 164.7 kJ/mol)
or from the emerging C-free H-carrier NH3 through ammonia dehydro-press1on
occur
downstream
by pressure-swing absorption and mechanical compressors. Efficiencies
typically improve
with
scalXXXXXX - XXXXX process over distributed
H2 production for energy-carrier applications.
(4).
H2 can also be !as Y-doped Ba2tures (300 °
to 8pure H2 from g;
!d electrochemically with proton (H+)-conducting
ceramic membranes such XXX
ons (BZCY), which are functional and stable over a
wide range of tempera ironments
(S.-lfl).
Proton ceramic electrochemical reactors (PCERs) extract XXX
XXX ically pumping protons across the membrane
(Ejg..J.A).
These offer process
intensification
(2)
by integi
-
g
reactions such as SMR+WGS or ADH with H2 separation and compression,
high energy efficiencies by supp·
lfil
;
heat electrically
(J),
and reduced CO2 emissions when that electricity is renewable.
(11).
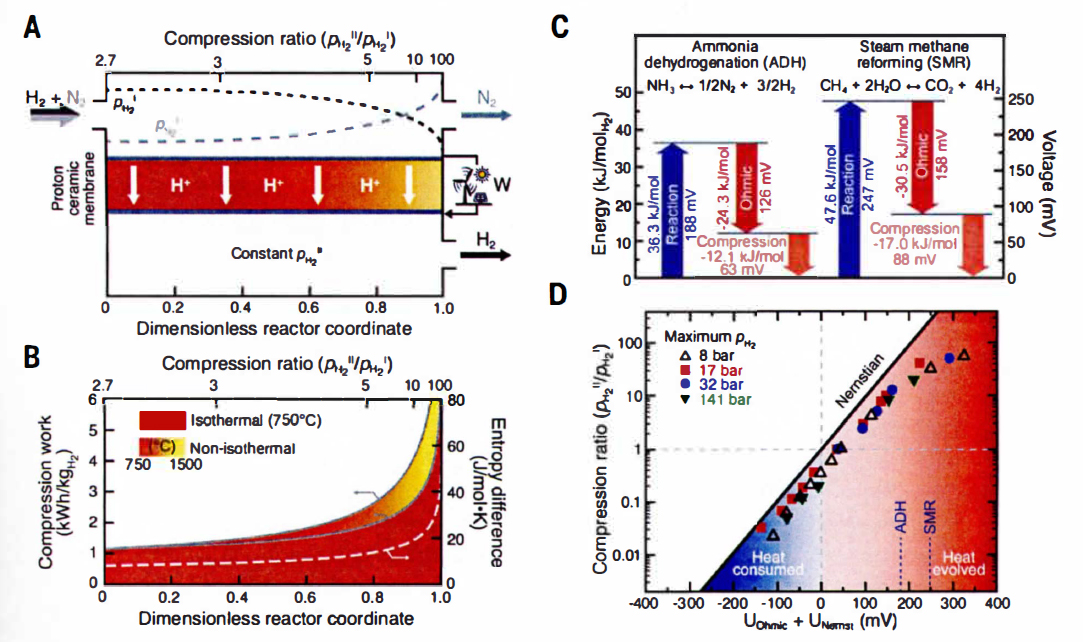
(A) Schematic of a proton ceramic
electrochemical continuous-flow reactor illustrating H2 separation
from an H2 + N2 mixture .
The local H2
compression ratio (top axis) increases as Pk,decreases upon H2
ex1raction along the reactor length (assuming a constant P}t), leading
to a corresponding temperature increase. (B) Compression work for
isothermal and non-isothermal H2 separation from an N2 + H2 mixture.
The local
compression ratio for H2 and the associated entropy difference L1S( x)
= R In (Pll,/Pk,
(x))
increase along the reactor
coordinate, which leads to an increase in compression work and heat
expelled from the compression process lw,,(x) = O(x) = T.O.S(x)]. If
left unbalanced, this heat increases the temperature throughout the
reactor, particularly in the latter parts, resulting in higher
compression work than would be ideal for isothermal operation. (C)
Energy balance and correlated voltages for thermally balanced
operating modes, which include reaction (ER
= liHR and UR= liHRlnF), charge transport (Ee= iRnF and
Uohmic = iR), and compression !Eco = UNernstnF and UNemst =
RT/nF In (Pll,/Pk,)1
for SMR+WGS at 750"C with an H2 compression ratio of 7.4 and NH3
cracking at 650"C with an H2 compression ratio of 5.4. See also fig.
S2 for a decompression mode of operation. (D) Compression ratio as a
function of Uohmlc + UNernst at 750"C for a maximum pH2 from 8 to 141
bars measured using representative PCER single cells (fig. S3) at ; =
50 mA/cm2, illustrating the different operation modes. The compression
ratio range was covered by adjusting the minimum pH2 as well as gas
flows to ensure a low degree of H2 extraction/dilution.
The blue region consumes and the red region evolves
heat.
As for any compression process, the work associated
with electrochemical H2 compression is minimized by operating
isothermally
(12).
In a
continuous-flow type of PCER, the compression ratio and associated
entropy difference
of H2 across the membrane increase with the extent of separation along
the reactor
(Fig..1B.
and fig.
S1). This entropy
difference is expelled as heat (Q
=
TvS) during the
compression process, which, if left unbalanced, leads to gradually
increasing temperature along the reactor and in tum larger electric
energy consumption per kilogram of compressed H2
(Fig..1.B.).
from compression with the extent or chemical reactions throughout a
stacked reactor poses one or the main hurdles in scaling XXXX
XX commercial scale. Furthermore, scaled
reactors with efficient current distribution
have of interconnect materials with high electrical conductivity and
chemical stability up to XXXXXX
thermal
expansion coefficient (8 x 10-6 K-1) of the preferred proton conductor
BZCY.
XXXX of reactor components can lead to mechanical stresses and electrical
contact XXXXX
We present an XXXXX aided by multiphysics
simulations and a new expansion-matched
metal/glass-ceramic XXXX (IC) enabling
deployable modular PCER stacks that retain the energy
efficiencies and H2 recovering XXXX single cells
( 9) while achieving a 36-fold increased H2 production capacity. The
reactor
is designed with gas XXXX that allow internal heat exchange from
exothermic to endothermic processes to minimize auxiliary heat input.
Our PCER can separate H2 by decompression while
recovering electric energy or by compression through supply of
electric energy ( Fig..l.D.)
at pressures up to 141 bars, illustrating the range of compression
ratios and associated cell voltages that can be achieved throughout
the PCER stack length. The PCER stack is a series of six barrels, each
with six single cells connected electrically in parallel
(Fig,2.A
and fig. S4A), that uses a newly
developed Ni-based glass-ceramic composite !Cs (13).
A conductive washer of the same material
is placed between the end of each membrane segment
and the IC plate
(Fig.2,
E and F).
Pure metals
with higher thermal expansions, such as
Ni or Cu, are prone to loss of electric
contact during thermal cycling (fig. S5A, Ni washer) because of an
expansion mismatch
with the IC, glass-ceramic, and membrane segment (fig. S58). Our
expansion-matched Ni/glass-ceramic
composite washer is applied in a partially heat-treated condition as a
sintered Ni/glass composite rather than a fully heat-treated
Ni/glass-ceramic composite. This means that the washer can deform
under the load applied during the heating phase of the sealing cycle
by virtue of viscous flow in the glassy matrix phase in the washer and
maintain intimate surface contact with both components.
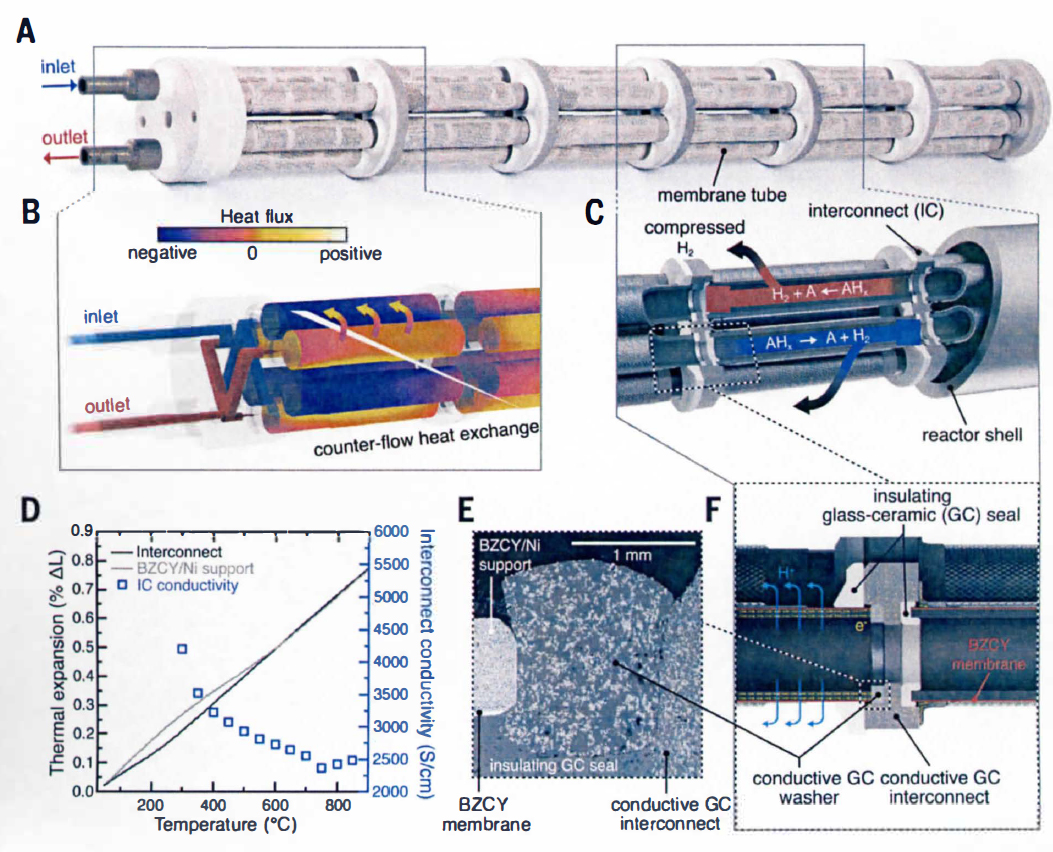
XXX
through the membrane and recovered as compressed H2 in
the outer chamber. (D) Thermal expansion upon cooling of the BZCY /Ni
support and the IC, and IC XXXXX of temperature. (E) Scanning
electron micrograph cross section of the interface between the BZCY/Ni
XXX a conductive glass-ceramic washer. (F) Schematics of IC and washer
assembly.
The glassy
XXXX phases in both the tubular cell
support and the IC and produces a mechanically XXXXX sealing cycle,
the glassy matrix phase in the washer crystallizes to produce a XXXX
composite bridge, which retains excellent electrical continuity
between the cell XXXX
thermal cycling. The adopted IC material exhibits conductivities 2500
S/cm at 750°C and XXXX expansion coefficients in a close-to-perfect
match with the thermal expansion of the membrane support XXXX and fig.
S5B), ensuring efficient current distribution throughout the stack and
mechanical robustness. The XXXX stable under reducing and CO2-rich
atmospheres, but can also be fitted with more oxidation
resistant
metallic components such as Ag for operation under oxidizing
conditions (fig. S5, C to E). The absence of Cr furthermore eliminates
degradation issues related to formation of resistive Cr203 scales or
evaporation of volatile Cr species during long-term operation at high
temperatures.
During operation, individual
cells will be net endothermic or exothermic depending on the degree of
reaction and H2 separation and compression throughout the stack length
(Figs.1D. and 2B), necessitating internal heat exchange. To guide the
optimal design of the stack, we adopted a three-dimensional multiphysics model integrating coupled gas flows, heat transfer,
current distribution, and reaction kinetics for SMR+WGS and ADH that
captures the behavior of the stack from single cell to stack level
(1.S). Our stack is designed with a U-bend type of gas flow pattern
achieved by a manifold that distributes the incoming gas to three of
the six gas channels in the stack while combining the three
corresponding exhaust streams. For the axial type design, the fast
kinetics of SMR and ADH concentrates the heat consumed by the
endothermic reactions to the initial segments of the stack, whereas
the heat caused by compression primarily evolves in the latter
segments. This leads to temperature increase along the reactor length
(Fig. 1A and .3, B and C) which in turn increases the cell Nernst
voltage and compression work (Fig, 3D). Our U-bend design, however,
mitigates this mismatch by spatially balancing the heat production
from compression with the heat consumption of the reactions enabling a
more uniform temperature profile (Fig.3A and Fig.S6, A and B). This in
turn lowers cell Nemst voltages and thus the required compression work
Fig.3F and Fig. S6C). Coupled with the high performing IC, the U-bend
design allows currents (i.e., hydrogen fluxes) to self-regulate
according to the local Nemst voltage (fig S4). For anhydrous ADH,
SMR+WGS and biogas this is particularly evident in the initial segment
where the reaction is concentrated because of fast kinetics (figs. S7
to S9). The slower reaction kinetics of aqueous ADH on the other hand
distributes the reaction over a larger portion of the stack (fig. S
10) .
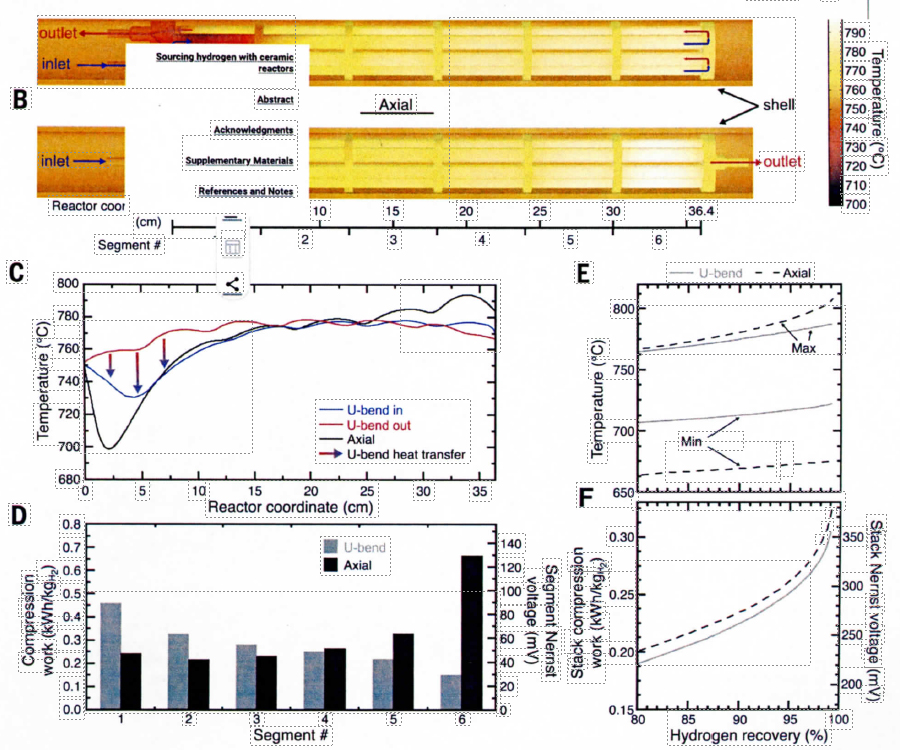
Fig. 3.
Multiphyslcs simulations of PCER stack thermally balanced operation.
Multiphysics simulations for a stack operating at 7so•c external
temperature with 20 bars of total pressure on both sides of the
membrane
and a mean current density of 0.60 A/cm2. Feed: 28.6% CH4 (0.597
NL/min), 71.4% H2O. Sweep: H2O (0.18 g/min). (A) Simulated temperature
fields in a U-bend PCER stack architecture, also showing the gas inlet
and outlet flow distribution. (B) Simulated temperature fields in an
axial PCER stack architecture. (C) Temperature profiles on the
reforming side in the axial and U-bend architecture along the reactor
length. The thermal balancing by heat transfer between first cell (net
endothermic) and last cell (net exothermic) for
U-bend PCER is
illustrated by vertical arrows. (D) Mean compression work and Nernst
voltage for each segment along the reactor length for the U-bend and
axial PCERs. The values were obtained by integrating the compression
work over each segment divided by the corresponding total flux or
current. (E) Effect of Hz recovery on the temperature distribution in
U-bend and axial PCERs. (F) Effect of Hz recovery on the mean stack
compression work and Nernst voltage for U-bend and axial PCERs.
To experimentally demonstrate
integration of reactions beyond SMR+WGS in our PCER stack (9), single
cells were
operated with NH3 in both anhydrous and aqueous form (.U). The cells
achieve >97% conversion of NH3 even at open-circuit conditions
(Fig.4.A) and near 100% conversions at high H2 recoveries thus leaving
an effluent stream virtually free of residual NH3. The cells
demonstrate comparable performance with anhydrous and aqueous NH3,
CH4, and biogas, retaining near faradaic behavior to above 0. 7 Ncm2
(fig. S11), reflecting the catalytic versatility of the porous Ni-BZCY
support. With CH4, the single cells even achieved >90% faradaic
efficiency up to 7.4 Ncm2 (corresponding to a H2 flux of 47 normal
milliliters-per-minute square centimeter) (fig. S12) thus doubling
the H2 production capacity to-date with these materials (9).
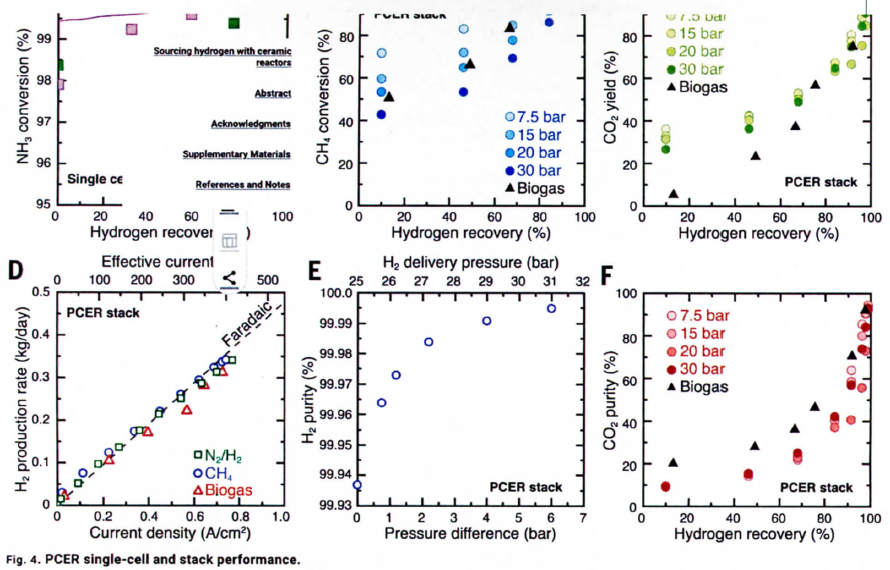
(A) NH3 conversion as a
function of H2 recovery, measured on a representative single cell
(fig.S3) at 650•c and 10 bars (pNH3 = 7.25 bars;
pH20 = 2.75 bars), and aqueous NH3 at 750·c and 10 bars (pNH3 = 3.1
bars; pH20 = 5.8 bars; and Piner1 = 1.1 bars). Purple and green lines
show the equilibrium conversion for NH3 and aqueous NH3, respectively.
(B) and (C) CH4 conversion and yield of CO2 versus H2 recovery,
respectively, of PCER stack at 750'C. (D) H2 production rate as a
function of applied current density for the stack with N2/H2 mixture
simulating
complete NH3 decomposition (75o•c. 1 0 bars), methane (8oo•c, 15 bars,
S/C = 2.5), and biog as (75o•c. 20 bars, S/C = 2.5). Effective current
is calculated as current density x PCER stack area (36 x 15 cm2) and
applied current as effective current/6 due to the series and parallel
electric architecture. (E and F) H2 purity (dry basis) versus H2 delivery
pressure and differential pressure across the membranes (E) and CO2
purity versus H2 recovery (F) for SMR+WGS in the stack at 75o•c.
Reforming side pressure= 25 bars, H2 side pressure= 25 to 31 bars.
Current density= 0.69 A/cm2.
The 36-cell PCER stack achieves nearly full CH4 conversion and high H2
recoveries (>99%; Fig.4 and figs. S13 and
Sl4) for CH4 and biogas, enabling complete equilibrium shift and a CO2
rich effluent stream for facile carbon capture. The series and parallel design of the stack facilitates an
effective aggregated current of up to 400 A (i > 0.73
A/cm2) with a H2 production rate up to 0.34 kg/day from CH4, 0.31
kg/day from biogas, and 0.34 kg/day from simulated fully decomposed NH3 streams (Fig.4D). We furthermore
demonstrate H2 compression to 31 bar with a purity of 99.995% (Fig.4.E)
facilitated for additional compression and use. The PCER stack shows
promising stability, retaining a H2 production rate of 2 normal liters
per minute after 1400 hours of operation (fig. S148). Both the H2
production rate [0.34 versus 0.025 kg/day (9)] and active area [584
versus 81 cm2 (14)] greatly surpass those of any reported for proton
ceramic applications. Moreover, the PCER stacks have demonstrated that
it is possible to deliver high-pressure H2 at high purity and a CO2
rich effluent at a hydrogen recovery and methane conversion >99%,
which is highly competitive with Pd-based membrane reformers (table
S2). These key performance indicators build the foundation for highly
energy-efficient hydrogen production at system level.
System modeling (13) of a 1 ton/day distributed H2 production plant
adopting our PCER stack (figs. S15 to S21l) reveals that efficiencies
of 91 % for CH4 and as high as 95% for anhydrous NH3 can be achieved
by virtue of microthermal integration and downstream heat recovery.
Furthermore, the PCER delivers a concentrated and pressurized stream
of CO2 when operated on methane or biogas Fig.4.E) that can be
purified and liquefied by cryogenic distillation, eliminating the need
for complex downstream absorption-based CO2 capture.
The high degree of process intensification achieved by our PCER stacks
enables a fuel-flexible energy-efficient alternative to established
technologies for distributed H2 production. Using a California 2020
electric grid carbon intensity scenario (82.92 gco2/MJeltec; see table S3 for references), H2
production with PCERs using CH4 as fuel would operate at lower
emissions (75.7 vs. 124. l gco2/MJH2) than water electrolysis powered
by grid electricity, even without CO2 sequestration. With decarbonization of the electric grid, CO2 sequestration is required
for methane re XXXXPCERs operated on 010gas even orrer tt2 proaucuon
w1tn net-negative caroon em1ss1on, as ctt4 from a XXXX process is XXXX
scenarios have used the CA GREET model (15) which includes fugitive
XXXX gas production that can be important (16).
To illustrate XXXX the PCER
technology, comparable well-to-wheel emissions for battery electric
vehicle XXXX engines (ICEs) with diesel fuel, and H2 fuel-cell
electric vehicles (FCEVs) are XXXX with sensitivity to electric
grid carbon intensity. In the California 2050 scenario, the
XXXX using H2 produced from CH4 with PCERs including CO2 sequestration
are 90% lower than XXXX with diesel fuel (145.4 gco2/km) and 26% lower
than FCEVs using H2 from grid-powered water XXXX (19.8 gco2/km).
NH3-based H2 can offer reduced emissions compared with on-site
grid-powered water XXXX (19.8 gc02/km). H3 based H2 can offer reduced
emissions compared with on-site electrolysis for a wide XXXX electric
grid carbon intensities, making FCEVs fueled with NH3 -based H2
directly comparable to BEVs in terms of CO2 emissions (6.3 gc02/km, a
reduction of 21 % compared with BEV in the California 2050 scenario).
Here, NH3 is assumed produced at off-site locations with favorable
renewable energy resources and transported as a liquid to the fueling
station where efficient ADH and separation to H2 takes place using
the PCER technology.
The growth of a new energy technology can be limited by access to raw
materials. A detailed examination of raw materials' usage of the PCER
stack (fig. S24) shows it is composed of non-precious, earth-abundant
materials, suggesting no material availability setbacks for scaling.
Acknowledgments
Funding: This work was supported by Norway's Ministry of Petroleum and
Energy through the Gassnova project CLIMIT grant 618191 in partnership
with Engie SA, Equinor, ExxonMobil, Saudi Aramco, Shell, and
TotalEnergies and the Research Council of Norway NANO2021 project
DynaPro grant 296548.
Author contributions:
Conceptualization: D.C., H.M.-F., T.P., P.K.V., T.S.B., J.M.S., C.K.;
Investigation: D.C., H.M.F., M.B., I.Y.-T., D.8., K.N., L.A., T.P.,
D.K.P., M.I.V., S.R.-B., T.S.B., C.K.; Methodology: D.C., H.M.-F.,
M.8., I.Y.-T., S. A., L.A., T.P., P.K.V., D.K.P., S.R.-8., C.K.;
Resources: M.B., D.B., K.N., M.I.V.; Software: I.Y.-T., S.A.;
Supervision:
T. N., T.P., f .M.S., C.K.; Writing - original draft: D.C., H.M.-F.,
M.B., l.Y.-T., S.A., P.K.V., D.K.P., T.N., T.S.B., J.M.S.,C.K.;
Writing- review and editing: D.C., H.M.-F., M.B., I.Y.-T.,
T.S.B., D.B., S.A., L.A., T.P., P.K.V., D.K.P., S.R.-B., T.N., T.S.8.,
J.M.S., C.K. Competing interests: D.C., H.M.-F., M.B., I.Y.-T.,
D.B., S.A., K.N., D.K.P., T.S.B., and C.K. are employed by CoorsTek
Membrane Sciences (CTMS). CTMS has filed relevant patent application
PCT/EP2017/076340. T.N. is a member of the CTMS board. l.Y.-T.'s
doctoral studies at the University of Oslo (UiO) are partially funded
by CTMS. The remaining authors declare no competing interests.
Data and materials availability: All data are available in the main
text or the supplementary materials. Experimental data are available
online at
http://hdl.handie.net/10251/181917.
Supplementary Materials
This PDF file includes:
Materials and Methods
Figs. S1 to S30
Tables S1 to S7
References (17-50)
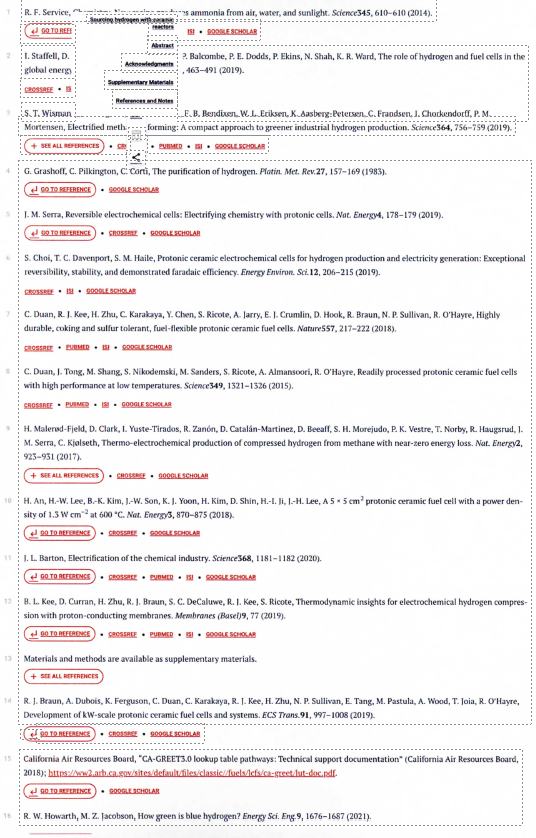
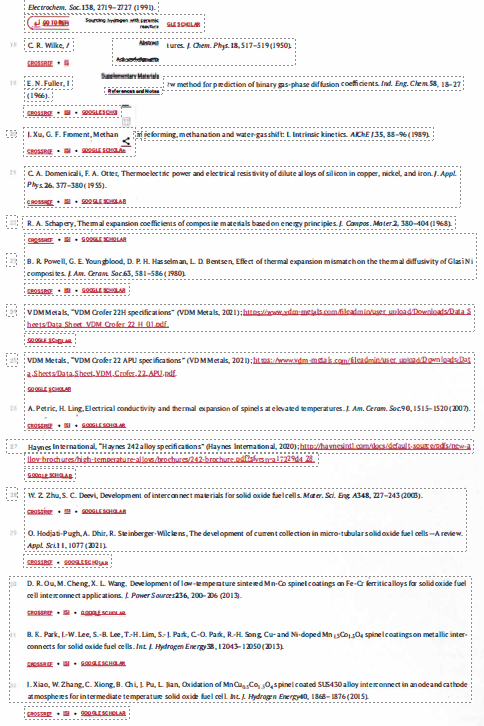
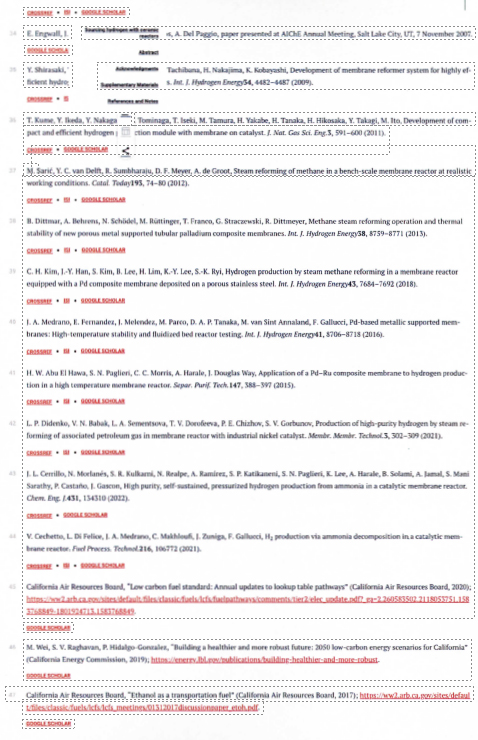
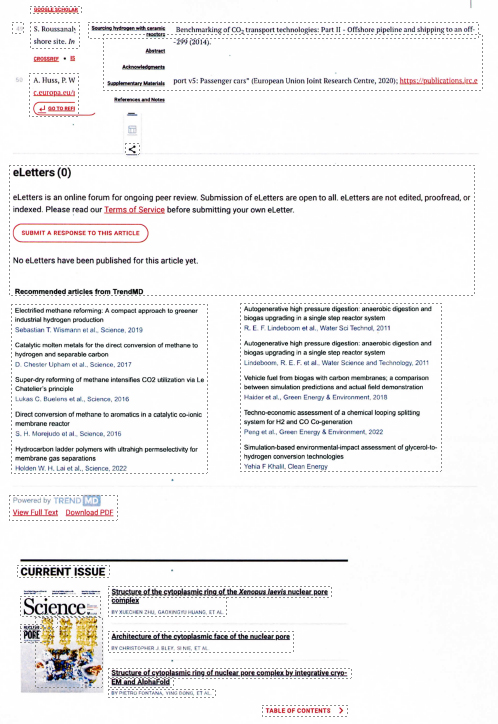
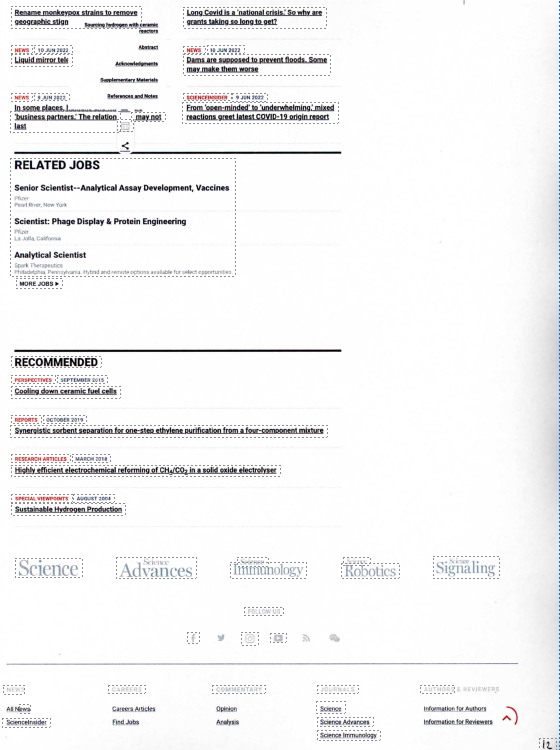
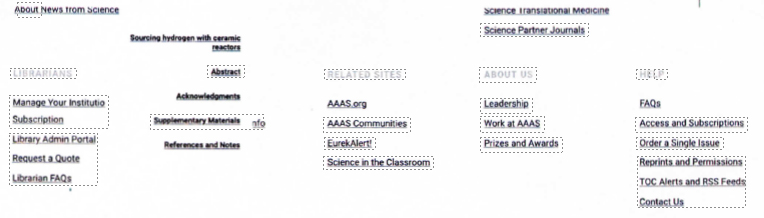
Rights and Disclaimers:
© April 23, 2022,
Exactrix®
Global Systems LLC. All rights reserved.
The information, services, and products are given to the user
with the understanding that neither the author(s), editor(s),
seller(s), or publisher(s) is engaged in rendering any legal,
business, or financial advice to the purchaser or the general
public. The views and opinions expressed are those of the author and
do not necessarily reflect the official policy or position of
Exactrix Global Systems, LLC (“Exactrix”); Green Play Ammonia; or
any other entity with which the author(s)/editor(s) have been, are
now, or will be affiliated with in the future.
Although we make strong efforts to make sure our information is
accurate, we cannot guarantee that all information in this article
or at the
exactrix.com site is always correct, complete, or up-to-date.
All products, product specifications, and data on the site is
subject to change without notice for any reason. Generally, changes
will be made to improve reliability, function, or design. Not all
products or product features will be available everywhere or at any
particular time. For legal reasons, features may be deleted from
products or we may refuse to offer products.
Any customer performance represented is historical. They are
individual experiences, reflecting real-life experiences of those
that have used our products and/or services in some way or another.
However, they are individual results and results may vary.
Testimonials are not necessarily representative of all of those who
will use our products or services. Any action you take based upon
this article or associated contents/sites is strictly at your own
risk.
This article provides critical commentary that is intended for
educational and informational purposes only. It may contain
copyrighted material whose use has not been specifically authorized
by the copyright owner. We believe this constitutes "fair use" of
copyrighted material as provided in section 107 of the US Copyright
Law. All product and company names are trademarks™ or registered ®
trademarks of their respective holders. Use of them does not imply
any affiliation with or endorsement by them. Any product names,
logos, brands, and other trademarks or images featured or referred
to are also property of their respective holders. They are not
affiliated with Exactrix, Green Play Ammonia, our sites, or
articles. They do not sponsor or endorse Exactrix, Green Play
Ammonia, our sites, or articles. If you wish to use this copyrighted
material for purposes of your own that go beyond "fair use," you
must obtain permission from the copyright owner. If you believe that
any content violates your intellectual property rights, please
notify us by email to
exactrix@exactrix.com.
Green Play Ammonia™, Yielder® NFuel Energy.
Spokane, Washington. 99212
www.exactrix.com
509 995 1879 cell, Pacific.
exactrix@exactrix.com
|